1. Introduction
Obesity is increasing dramatically and has already become a major clinical challenge for healthcare systems worldwide [1]. Obesity is a multifactorial disease, influenced by both genetic and environmental factors. The onset of obesity is due mainly to low energy expenditure (e.g., from exercise) combined with high caloric intake. This leads to an excessive accumulation of fat in the adipose tissue, accompanied by low-grade inflammation, hypoxia and oxidative stress.
Oxidative stress is defined as an imbalance between the reactive oxygen species (ROS) scavenging and producing systems in the organism. ROS include molecules, such as hydrogen peroxide (H2O2), superoxide (O2•−) and the hydroxyl radical (OH−). The controlled production of these molecules is known to help protect against microorganisms during infectious processes, as well as contribute to normal functions in the cell, including proliferation, differentiation and signaling [2]. However, a non-physiological increase in ROS levels from excessive caloric intake, inflammation or hypoxia, or a decrease in the antioxidant capacity of the organism can lead to the aforementioned alterations.
The antioxidant defense system maintains ROS homeostasis in the cells. It comprises both endogenous and exogenous antioxidants. Endogenous antioxidants include enzymes that degrade ROS at different levels and in different compartments inside and outside of the cells, such as glutathione peroxidases (GPXs), catalase (CAT), paraoxonases (PONs), superoxide dismutases (SODs), peroxiredoxins (PRDXs), glutathione reductase, thioredoxin reductase, heme-oxygenase 1, cytochrome c oxidase, as well as methionine sulphoxide reductase involved in the repair of oxidized proteins, xanthine oxidase, a drug metabolizing enzyme, and the cytochrome c oxidase complex, which regulates the electron transport chain. In addition, some of these enzymes require endogenous cofactors, such as glutathione and lipoic acid, in order to perform their ROS scavenging activities. Exogenous antioxidants include vitamins, carotenoids, polyphenols and trace elements, such as selenium and zinc, reviewed in [3].
It is known that genetic variations, such as single nucleotide polymorphisms (SNPs), can affect the functioning of antioxidant enzymes and increase the risk of certain diseases, such as cancer [3]. However, to our knowledge, the impact of genetic variations in the genes associated with oxidative stress regulation has not been fully studied nor reviewed in the context of obesity. Detailed studies in this field could clarify the mechanisms involved in the development of the comorbidities of obesity, such as metabolic syndrome and insulin resistance, even in the development of obesity itself [4]. Thus, the aim of this review is to summarize the current knowledge about the association of genetic variations in antioxidant defense system genes, oxidative stress producing systems and related transcription factors with obesity risk and phenotypes.
In the future, the characterization of these SNPs in obese patients could contribute to the development of controlled antioxidant therapies that would be potentially beneficial for the prevention and treatment of obesity and its derived metabolic complications.
Go to:
2. Methodology
We conducted a systematic review of the literature by using the PubMed database. The following phrases were included in the process: (1) “obesity” AND “polymorphism” AND “oxidative stress”, limited to human studies, gave 47 results; (2) “obesity” AND “polymorphism” AND “oxidative stress”, limited to animal studies, gave eight results; (3) “obesity” AND “gene expression” AND “adipose tissue” AND “oxidative stress”, limited to human studies, gave 28 results; (4) “obesity” AND “gene expression” AND “adipose tissue” AND “oxidative stress”, limited to animal studies, gave 71 results; (5) “obesity” AND “mechanisms” AND “gene” AND “oxidative stress”, limited to human studies, gave 31 results; and (6) “obesity” AND “mechanisms” AND “gene” AND “oxidative stress”, limited to animal studies, gave 30 results. A total of 215 results in English were obtained, and titles and abstracts were revised to select a total of 73 articles that were read in full. We included articles that had the clear aim of investigating the role of SNPs or genes on the risk of obesity or its metabolic complications. In addition, previous reviews focusing on conditions other than obesity were carefully examined in order to better characterize the role of antioxidants in the context of disease. Additional articles not found in this search were identified by exploring references in key articles, as well as by individual searches of specific genes.
Go to:
3. Oxidative Stress in Obesity
The adipose tissue is an endocrine organ that produces a variety of molecules, including adipokines, such as adiponectin and leptin, and cytokines, such as tumor necrosis factor alpha (TNFα) and interleukins 1β (IL-1β) and 6 (IL-6) [5,6]. It is well known that adipose tissue in obese individuals undergoes many pathological changes, due to the accumulation of fat, such as inflammation, hypoxia and increased oxidative stress [7,8]. Upon the accumulation of excessive fat, the adipokine secretion profile becomes altered and peripheral tissues are affected, contributing to the appearance of health problems, such as dyslipidemia, hypertension, insulin resistance, diabetes and atherosclerosis (Figure 1).
Figure 1.
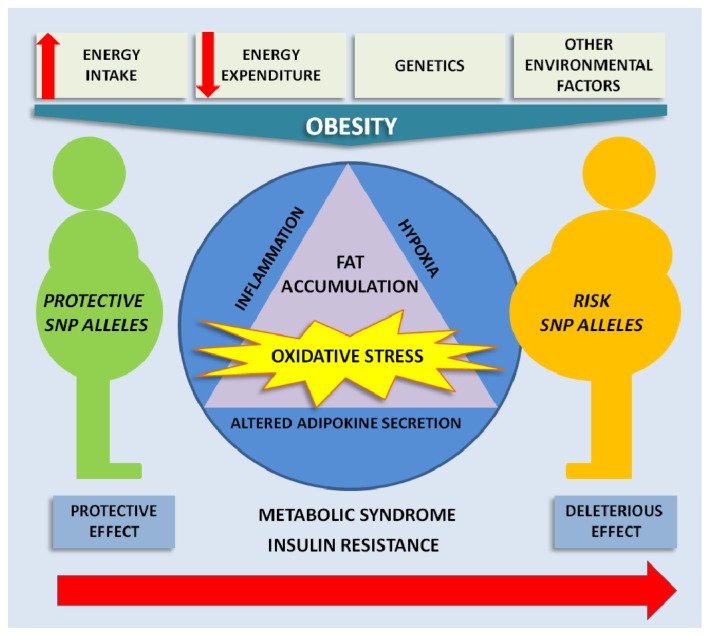
A schematic diagram of the multifactorial character of obesity. These factors influence the development of obesity and its associated comorbidities by altering adipokine secretion, hypoxia and inflammation with associated oxidative stress. The presence ...
The higher fat and carbohydrate intakes associated with obesity may be responsible in part for the enhanced ROS production, due to the saturation of the electron transport chain. Free fatty acids (FFAs) have had this effect in mouse models [9], and in humans, FFAs generate high H2O2 levels in the mitochondria [10]. Thus, the link between obesity and enhanced oxidative stress might be due to the hyperglycemia, high circulating FFA, decreased antioxidant defenses and chronic inflammation associated with obesity. Indeed, in obese humans, indicators of cellular and systemic oxidative stress have been found in many studies (Table 1). Levels of plasma thiobarbituric acid reactive substances (TBARS) and urinary 8-epi-prostaglandin F2α (8-epi-PGF2α) were augmented in obese individuals [11,12]. A study conducted in severely obese children found similar results, with higher 8-isoprostane F2α and malondialdehyde (MDA) plasma concentrations, as well as increased nitric oxide production, as reflected by higher nitrite, nitrate and nitrotyrosine values, in obese children [13]. The activities of antioxidant enzymes, such as glutathione peroxidase and catalase have also been observed to be lowered in obesity, as will be described below [14,15].
Table 1.
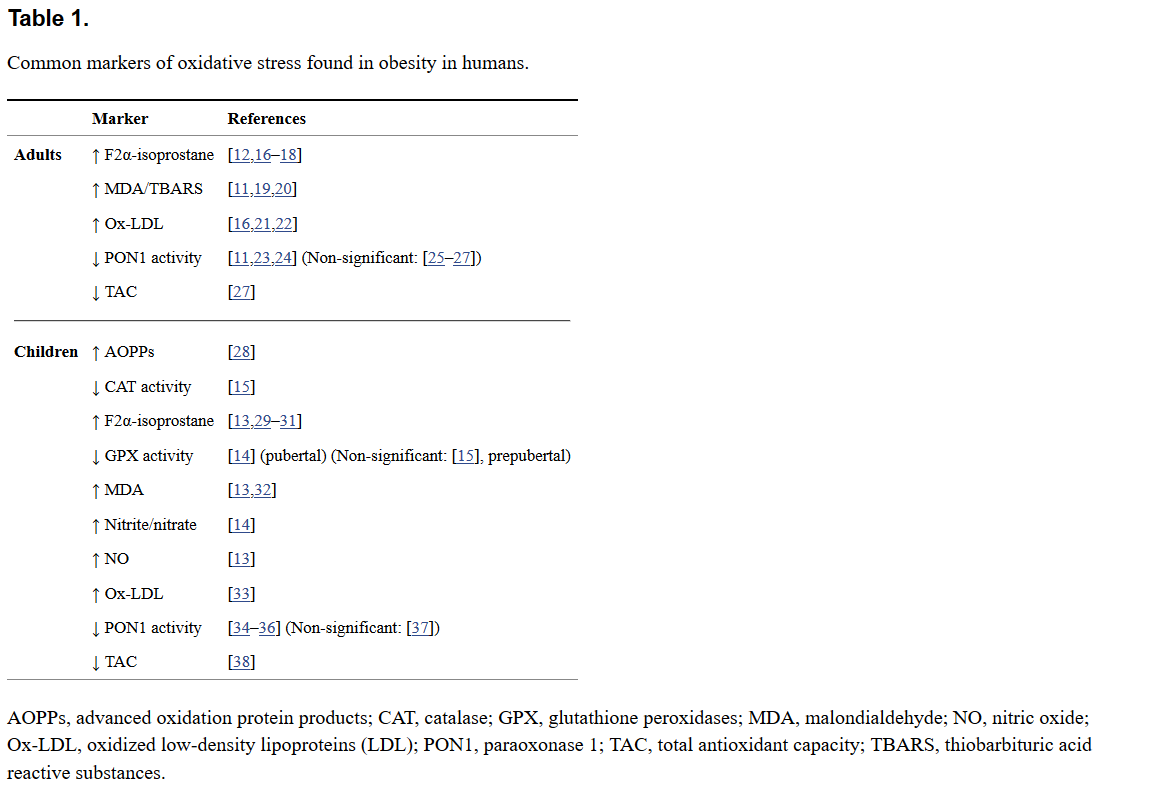
Common markers of oxidative stress found in obesity in humans.
In addition to ROS produced by caloric intake, cells also have ROS-producing systems for physiological processes, including protein folding in the endoplasmic reticulum, xenobiotic metabolism, DNA metabolism and the nicotinamide adenine dinucleotide phosphate-oxidase (NADPH oxidase) complex that produces ROS in response to insulin and cytokines (like TNFα) as part of a signal-transducing system [39]. The alteration of these systems can generate additional quantities of ROS and contribute to higher oxidative stress levels.
The enhanced oxidative stress associated with obesity leads to the oxidation of proteins, lipids and DNA and, eventually, to alterations in the modulation of gene expression and signaling pathways [40]. Indeed, these alterations in cellular and tissue components contribute to chronic inflammation and, thus, to the development of diseases, such as obesity and insulin resistance [41,42]. In fact, chronic oxidative stress is known to induce inflammation, and obesity is considered a disease of chronic low-grade inflammation [43]. In addition, ROS are a key element in the adipogenic process, and the possibility exists that their excessive increase helps in the development of obesity by a stimulation of adipogenesis [4,44].
The role of transcription factors in the development of obesity comorbidities is gaining attention. Peroxisome proliferator activated receptor gamma (PPARγ) and PPARγ coactivator-1α (PGC1α) are very well-known transcription factors in the adipose tissue. Some studies have suggested that PPARγ is implicated in cellular responses against oxidative stress [41,45,46], whereas the role of PGC1α in the induction of the expression of ROS detoxifying enzymes has been better characterized [47,48]. In the present review, we will focus on the known variations in these genes and go through the findings concerning their impact on the risk of obesity insulin resistance. Nuclear erythroid factor 2-like 2 (NRF2), one of the most important transcription factors in the oxidative stress response, will also be considered with respect to its role in ROS responses and obesity.
Go to:
4. Enzymatic Antioxidant Defense Genes
4.1. Glutathione Peroxidases
The GPX family is composed of at least eight isoenzymes in mammals and constitutes one of the main antioxidant defense systems, using glutathione to degrade H2O2 [49]. GPX1 is the most abundant isoenzyme, ubiquitous in the intracellular fraction and formed by four 22-kDa subunits, each carrying one selenocysteine. GPX2 is the gastrointestinal form, and GPX3 circulates in blood and is secreted from the kidney [50]. GPX5 and 6 are specific from the epididymis and the olfactory epithelium, respectively. Finally, GPX 4, 7 and 8 are the earliest in evolution, as they share sequences with protozoa and invertebrates [51]. GPX4 is a membrane-bound form, important for spermatogenesis. Interestingly, GPX7 (also non-selenocysteine-containing phospholipid hydroperoxide glutathione peroxidases or NPGPX) and GPX8 have no clear enzymatic activity. However, the endoplasmic reticulum enzyme, GPX7, expressed in adipocytes and their precursors, was shown to act as an oxidative stress sensor/transducer in the regulation of ROS accumulation [52].
In animal studies, cellular and extracellular GPX activity was shown to be lower in the adipose tissue of obese rats [53]. In a study carried out with obese mice, the mRNA expression of GPX1 was increased after caloric restriction [54]. However, GPX1 knockout mice are protected against high-fat diet-induced insulin resistance and atherosclerosis [55–57]. Concerning GPX3, in a study involving obese mice, GPX3 expression was found to be selectively decreased in adipose tissue and plasma; the same effect was achieved by treating mice with either both TNFα or hypoxia. The use of antioxidants and the anti-diabetic drug, rosiglitazone, both succeeded in restoring GPX3 expression in adipose tissue, improving the insulin resistance phenotype and attenuating the inflammatory gene expression pattern [58]. Another study that supports GPX3 activity against obesity showed that estrogen receptor α activates GPX3 transcription and that this mediates its fat mass reducing effects [59]. In the case of GPX7, an elegant study showed that the loss of GPX7 enhances oxidative stress [52] and adipocyte hypertrophy and increases white adipose tissue mass by stimulating adipogenesis [4] in mice. Finally, in human studies, pubertal obese children with insulin resistance showed lower erythrocyte GPX activity than the control group [14], whereas no changes were observed in prepubertal obese children [15].
Regarding genetic variations, SNPs associated with obesity and insulin resistance have been described for the GPX1 and GPX7 genes (Figure 2, Table 2). The GPX1 gene harbors a well-known missense polymorphism (C to T substitution) at nucleotide 594 that results in the substitution of leucine for proline at codon 198 of the protein (Pro198Leu; rs1050450). Many studies have shown the Leu allele to be associated with worse outcomes for oxidative stress, central obesity and insulin resistance, with some sex-related differences. Male Leu allele (T) carriers had higher metabolic syndrome prevalence, demonstrating higher waist-hip ratios, triglycerides (TAG), insulin, homeostasis model assessment of β-cell function (HOMA-β) and systolic and diastolic blood pressures [60]. Women carrying the same allele showed higher body fat mass, insulin and homeostasis model assessment of insulin resistance (HOMA-IR). In an intervention study, the authors showed that nutritional supplementation with selenium from Brazil nuts was associated with higher DNA damage in Leu carriers [61]. Carriers of the Leu allele have also been shown to have significantly higher levels of lipoperoxides and MDA in low-density lipoproteins (LDL) [62]. It was shown that the combination of Pro198Leu SNP with the copy number variant (CNV) Ala5/Ala6 at codon7–11 decreases the activity of the enzyme by 40% in vitro [63]. In the same study, it was demonstrated that the combination of two other SNPs (−602A/G, 2C/T) decreased the transcriptional activity of GPX1 by 25%. These data suggest that the Leu allele is associated with lower GPX activity and a subsequent higher oxidative stress from ROS, thus generating worse outcomes in obesity-associated phenotypes.
Figure 2.
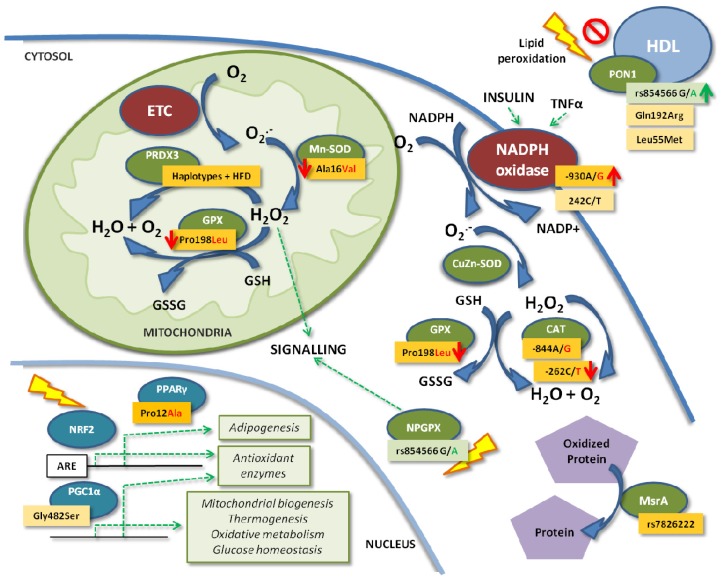
Effects of the main known SNPs of the antioxidant defense system genes in the cell on obesity. Dark orange and green boxes indicate higher or lower obesity risk associated with the SNP, respectively. Light orange boxes indicate conflicting results. Red ...
Table 2.
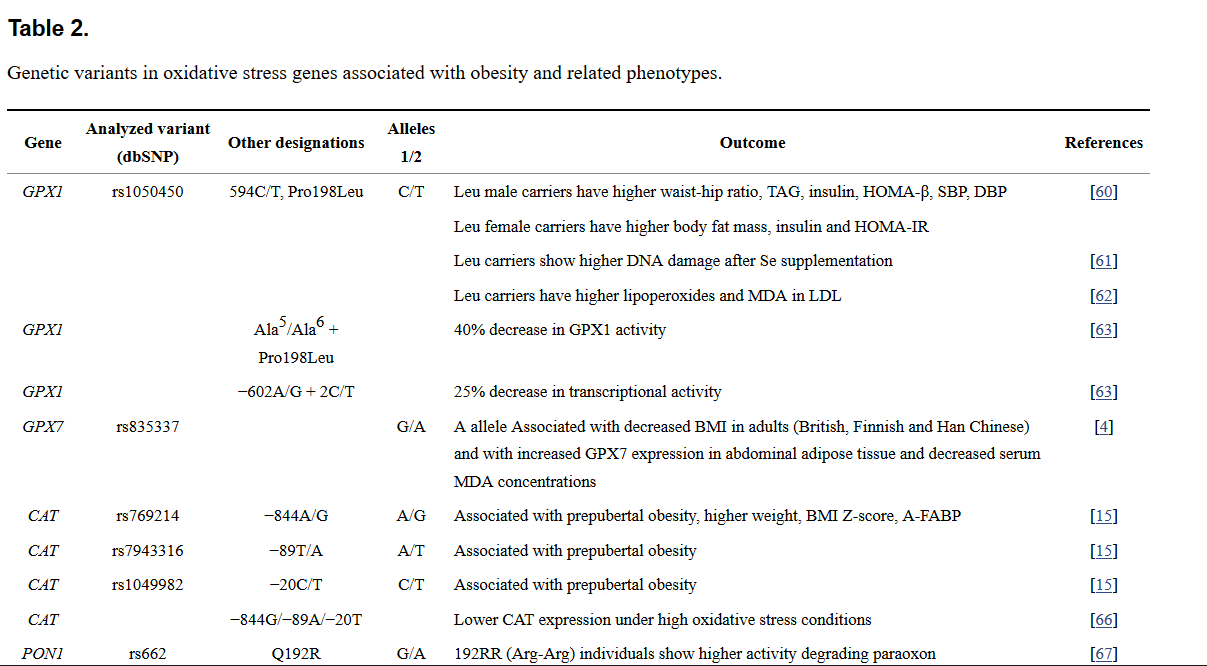
Genetic variants in oxidative stress genes associated with obesity and related phenotypes.
In the case of GPX7, SNPs near this gene have been associated with lower GPX7 expression and increased adiposity in several populations. The variant, rs835337 (G/A), located upstream of the gene, was associated with body mass index (BMI) in adults. The minor allele (A) of this genetic variant was associated with decreased BMI and increased GPX7 expression in abdominal adipose tissue together with decreased serum MDA concentrations. In this way, the SNP confers protection against obesity potentially by a decrease in ROS levels. The proposed mechanism is that adipogenesis is impaired by the presence of lower ROS levels, needed for the normal adipogenic process [4]. In summary, GPXs with enzymatic activity seem to be involved in the protection against obesity-derived metabolic complications, whereas the sensor/transducer GPX7 regulates responses against ROS in the earlier steps of obesity development.
4.2. Catalase
CAT is one of the most important antioxidant enzymes in the cell, located in the peroxisomes. It degrades any H2O2 that exceeds the physiological levels. CAT expression was increased after caloric restriction in the adipose tissue of obese mice [54]; however, its expression in mice hearts has also been observed to be increased after 30 weeks of high-fat feeding, possibly to compensate for the observed significant decrease in CAT-specific activity [64]. Moreover, CAT erythrocyte activity was lower in children with insulin resistance and obesity [15,65].
Its genetic variation has traditionally been studied for diseases other than obesity. Due to this fact, our group recently published a study conducted on obese children that showed the association of some SNPs located in the CAT promoter with obesity [15] (Figure 2, Table 2). We found that the presence of the rare SNP variants, rs769214 (−844A/G), rs7943316 (−89T/A) and rs1049982 (−20C/T), was significantly and positively associated with prepubertal obesity. All of these SNPs were in linkage disequilibrium (LD) and formed a haplotype that had been previously described as associated with lower CAT expression in human cell lines under high oxidative stress [66]. The association between these SNPs and the principal insulin resistance and obesity markers was also studied, and we found that SNP rs769214 is associated with significantly higher weight, body mass index (BMI) Z-score and adipocyte fatty acid-binding protein (A-FABP), as well as with a higher plasma insulin concentration (not significant), without any observed effect on erythrocyte CAT activity. Another variant investigated was the SNP rs1001179 (−262C/T) in the 5′ untranslated region (UTR) of the CAT gene. Several studies demonstrated that the T allele was associated with lower CAT enzyme activity [96–98], whereas those results were rejected by others [99].
These results suggest that CAT activity and expression are involved in the defense mechanisms against obesity-derived metabolic complications. The presence of the described SNPs could lead to lower CAT transcriptional activity and, thus, to lower CAT expression levels and activity in the cell. This would further contribute to cellular oxidative stress and its effects on cell dysfunction by altering signaling cascades or increasing the damage to macromolecules by oxidation.
4.3. Paraoxonases
The PON family consists of three antioxidant isoenzymes. PON1 and PON3 are expressed mainly in the liver and kidneys and are found bound to high-density lipoproteins (HDL) in the circulation. They inhibit the lipid peroxidation of the LDL and HDL particles in plasma. PON2 is a more ubiquitous membrane-bound form, found in a variety of tissues. Regarding alterations in PON expression in obesity, only one study has been conducted, in pigs, where PON3 mRNA expression in fat tissue was positively correlated with subcutaneous, visceral and total body fat weight, indicating a potential role for PON3 in obesity [100].
In the case of PON1 activity in the context of obesity, some studies have found decreased paraoxonase and arylesterase activities in the obese [11,23,24], whereas others have not [25–27]. Similar findings have been observed in children, with some authors observing altered PON1 activities [34–36], and others finding no PON1 activity changes in obesity in children [37].
PON1 activity is known to be influenced by environmental factors, such as age, diet or medications, but the main cause of variation is genetics [101]. Two missense SNPs in the PON1 gene, Q192R (rs662) and L55M (rs854560), have been traditionally studied (Figure 2, Table 2). Individuals with the 192RR (ArgArg) genotype have higher paraoxon-degrading activity [67], while 55LL (LeuLeu) individuals exhibit increased serum PON1 concentrations [102]. A study conducted in Portuguese women showed an association of the R allele with a higher risk of obesity [26], whereas a study in Mexican adults revealed no association between the variant and risk of obesity [25]. Another study failed to find any association of the SNP with obesity in adolescents [68]. Our group recently published a study carried out in prepubertal children that also confirmed the lack of an effect of Q192R on childhood obesity risk [37]. In this study, we described a novel PON1 SNP, rs854566, which was found to be associated with protection from obesity in children, perhaps due to an observed increase in PON1 lactonase activity, although PON1 activities did not show any differences between obese and normal-weight subjects (Figure 2).
4.4. Peroxiredoxins
PRDXs are a family of six thioredoxin-dependent peroxidases that degrade H2O2 in the cell. Recent studies have clearly shown that PRDXs contribute to ROS signaling, regulating cell proliferation, differentiation and apoptosis. PRDX3 is located exclusively in the mitochondria, where it scavenges up to 90% of the H2O2 produced in this organelle, followed by scavenging by GPX1 and GPX4 [103]. Taking into account that mitochondrial respiration is the principal ROS producer, PRDX3 is considered highly important in terms of antioxidant defenses and redox status regulation.
PRDX3 levels have been observed to be decreased in the adipose tissue of obese mice and humans [44]. In the same study, PRDX3 knock-out mice exhibited increased fat mass, as well as increased adipogenic and lipogenic gene expression in adipose tissue, leading to an obese phenotype. Additionally, increased O2•− levels and protein carbonylation were observed in mitochondria, together with defects in mitochondrial biogenesis. In addition, adiponectin was downregulated, and plasminogen activator inhibitor (PAI) was upregulated, in accordance with the presence of impaired glucose tolerance and insulin resistance observed in PRDX3 knock-down adipocytes.
The impact of PRDX3 genetic variations on obesity has been investigated in only one nutrigenomic study, in which it was found that four SNPs in the PRDX3 gene and the haplotype they formed were associated with higher BMI and obesity in Japanese people, when combined with a high-fat diet (HFD) [69] (Figure 2, Table 2). The SNPs, rs3740562 (A/G), rs2271362 (C/T), rs7768 (G/C) and rs3377 (A/C), were significantly associated with BMI after a multiple testing Bonferroni correction, whereas rs1553850 (A/T) was not. The haplotypes, A-A-T-G-A and T-G-C-C-C, also showed a significant association with decreased and increased BMI, respectively. As an HFD induces ROS production, Hiroi et al. investigated its possible role in these associations. The study of the interactions between the genotypes and haplotypes and dietary fat intake revealed that these genetic associations could only be observed in the group with the high-fat intake. Moreover, the association of the genotypes with higher BMI was observed only in the high-fat intake group. Altogether, these findings indicated a role for PRDX3 genetic variations and fat intake in the modulation of BMI and obesity risk.
More studies are needed to investigate the effects of these and other SNPs on enzyme levels and activities to elucidate the link between their presence and a higher risk of obesity. One hypothesis could be that, under a high-fat diet, PRDX3 could be saturated by the excessive ROS produced in the electron-transport chain. Further studies could help in defining the role of this haplotype, which perhaps decreases PRDX3 expression or activity, explaining the aforementioned findings.
4.5. Superoxide Dismutases
The three members of the SOD family are the first line of defense against ROS, eliminating the strong superoxide radical and producing H2O2 that can then be degraded by CAT, GPXs and PRDXs [104]. CuZn-SOD (SOD1) is a homodimer localized in the cytosol. Mn-SOD (SOD2) is a tetramer localized in the mitochondria, and the extracellular tetramer CuZn-SOD (SOD3 or EC-SOD) is localized exclusively in extracellular spaces. MnSOD is one of the most important antioxidant enzymes, because most superoxide is produced in the mitochondria.
EC-SOD levels have been observed to increase in the white and brown adipose tissue and in the plasma of obese mice. In the same study, TNFα and IL-1β levels were also observed to be higher in white adipose tissue, which could be interpreted as an adaptation by the adipose tissue to the enhanced oxidative stress associated with obesity [105]. However, in a study of type 2 diabetic patients, EC-SOD levels were shown to be reduced and inversely related to BMI and HOMA-IR [106]. Interestingly, overexpression of SOD1 or SOD2 in mice reduced oxidative stress in vivo [107] and protected mice against high-fat diet induced glucose intolerance and insulin resistance, but not against obesity [108,109]. In contrast, another study showed restricted growth for SOD1 knockout mice, indicating a malfunction in absorption, as seen by the accumulation of lipid droplets in enterocytes [110].
The best-known SOD2 SNP is rs4880 (C/T). It is located in the second exon, and its presence generates a change in the 16th amino acid in the mitochondrial targeting sequence of the protein from alanine to valine [111] (Figure 2, Table 2). The Val-MnSOD variant has been associated with the arrest of MnSOD in the inner membrane and lower MnSOD homotetramer formation in the mitochondrial matrix, together with a lower efficiency of the enzyme in dismutating O2•− into H2O2 [80]. Strikingly, both alleles in their homozygous form have been found to be associated with an increased risk for a variety of diseases, perhaps due to the increased O2•− or H2O2 levels in ValVal and AlaAla subjects, respectively. However, only the Val allele was found to be associated with a higher risk of obesity in the elderly [81]. In addition, the presence of the ValVal genotype was related to higher levels of pro-inflammatory cytokines, such as IL-1, IL-6, TNF-α and interferon gamma (IFN-γ), and lower levels of IL-10 [82]. In contrast, Val allele carriers from a healthy cohort showed lower baseline levels of DNA damage [83]. Another study found that the ValVal genotype was more frequent among obese children with non-alcoholic steatohepatitis than those without the disease, although this difference was not significant [112].
These findings indicate that the role of superoxide in the origin of obesity-derived alterations should be investigated. Moreover, these data support MnSOD as an essential enzyme in the regulation of ROS, the levels of which need to be perfectly balanced so that metabolic complications are avoided.
4.6. MsrA
The methionine sulphoxide reductase A (MsrA) is a 26 kDa protein localized in the cytosol and mitochondria involved in the antioxidant defense repairing of oxidized proteins, specifically by reducing the methionine-S-sulphoxide epimers back to methionine, using thioredoxin as a cofactor. Protein oxidation is a reversible process that has been proposed as one of the mechanisms by which oxidative stress leads to metabolic alterations, such as insulin resistance [113,114]. Due to this fact, the alteration of protein repairing enzymes, such as MsrA, is thought to be a key potential disruptor of oxidized protein-based signaling regulation. In a study conducted in wild-type and high-fat diet-fed rats, Uthus and Picklo observed a reduction in MsrA activity of 25% in visceral adipose tissue in a tissue-specific manner [115]. Additionally, more recently, Styskal et al. showed that high-fat diet-fed MSRA−/− mice develop a more severe insulin-resistant phenotype with parallel reduced insulin signaling compared to wild-type mice [42].
Moreover, several loci in the MSRA gene have been associated with visceral obesity (Figure 2, Table 2). The first SNP to be associated with obesity was rs7826222 (also named rs545854), found to be positively associated with waist circumference (WC) in a genome-wide association study (GWAS) meta-analysis conducted in adult Europeans [70]. This finding was confirmed in other studies in Caucasians [71] and Hispanic women [72]. Yeung et al. also found the minor allele C to be associated with an increase in type 2 diabetes risk in men [73]. However, other studies did not observe a significant association between the variant rs7826222 with BMI in Chinese women [74] or with metabolic syndrome [75] or visceral fat [76] in Japanese. Other described MSRA SNPs are rs473034 and rs516175, associated with extreme childhood obesity [77] and the BMI Z-score in Singaporeans [78], respectively. In another study, Scherag et al. found no effect of three genetic variants near the MSRA gene (rs13278851, rs17150703 or rs516175 on a one-year lifestyle intervention to reduce weight in overweight children and adolescents [79]. Further studies investigating the potential role of these genetic variants in MsrA activity or expression are needed.
Go to:
5. The ROS Producer: NADPH Oxidase
One of the most important ROS producers in cells is the NADPH oxidase complex, which generates O2•− and, subsequently, other ROS, such as H2O2, during the phagocyte respiratory burst [116]. However, its activity is not limited to phagocytes, as other cells use NADPH oxidase-generated ROS as signaling mechanisms. It is known that insulin and cytokines act on this enzymatic complex, stimulating H2O2 production and providing a link between ligand binding and the intracellular redox state contributing to intracellular signaling cascades [39]. This complex is formed from six subunits, p22phox and gp91phox, which form cytochrome b558, p47phox, p67phox, p40phox, and rac. This enzymatic complex generates free radicals from oxygen and NADPH.
The SNP −930A/G in the promoter of the p22phox gene was found to be associated with higher p22phox expression and NADPH oxidase activity in phagocytic cells from hypertensive patients carrying the GG genotype [84] (Figure 2, Table 2). The higher NADPH oxidase activity resulted in higher ROS production, which, in turn, increased the risk of insulin resistance [117]. Along these lines, the GG genotype was associated with higher HOMA-IR and insulin, but not with obesity, in a cohort of obese and normal weight Spanish subjects [85]. Another SNP in the p22phox subunit is 242C/T (Figure 2). Japanese type 2 diabetic patients carrying the T allele of this SNP showed a significantly lower intima media thickness (IMT) and lower 8-hydroxy-2′-deoxyguanosine (8-OHdG) values (not significant), whereas the non-diabetic T allele carriers were protected against insulin resistance, exhibiting lower HOMA-IR and fasting plasma insulin values [86]. However, another study reported that the CC genotype conferred protection against diabetes mellitus and obesity and was associated with lower fasting plasma glucose levels and waist circumference in hypertensive patients [87]. Other studied SNPs include rs7195830 (C allele) and rs12709102 (T allele), which were associated with a higher risk of obesity in women [88].
All of these findings taken together indicate that higher NADPH oxidase activity, and the concomitant ROS production could act in modulating the insulin signaling pathway. The genetic variations in the genes of NADPH oxidase subunits should be further investigated to better understand their impact on enzymatic activity and the consequences on insulin signaling. This phenomenon could be a link between obesity and insulin resistance. Knowing the genotype of obese patients could help in treating them against further damaging metabolic complications.
Go to:
6. ROS Response Mechanisms: Transcription Factors
6.1. PPARγ
The nuclear hormone transcription factor, PPARγ, regulates adipogenic differentiation and lipid metabolism. Its expression is increased in the adipose tissue of obese individuals [118,119]. It binds to lipophilic ligands, such as poly-unsaturated fatty acids, prostaglandin derivatives and oxidized fatty acids [120]. Some studies have shown that PPARγ plays a role in the regulation of the antioxidant response to ROS, although the results are divergent. In a study in mice, it was shown that the adipose tissue-specific loss of an allele of PPARγ, with the subsequent loss of activity, was associated with more resistance to paraquat-induced oxidative stress. This was at least partially mediated through the upregulation of ROS scavenging genes, including GPX1, glutathione reductase, PRDX3, SOD2 and CAT, and the upregulation of the ROS responding transcription factor FOXO3A in adipose tissue [45]. This study concluded that reduced PPARγ activity in adipose tissue has beneficial effects. However, in another study, the authors showed that the activation of PPARγ by its ligands decreased TNFα or glucocorticoid-induced ROS production in human adipocytes [41]. In a previous study, Itoh et al. hypothesized that oxidative stress could exert some of its effects on intracellular signaling through PPARγ, and indeed, they showed that PPARγ expression was downregulated by H2O2, TNFα and lysophosphatidyl choline, which is the major constituent of oxidized LDL [46].
The best-known SNP in the PPARγ gene is rs1801282, which generates an amino acid change in the protein at codon 12 from proline to alanine (Pro12Ala) (Figure 2, Table 2). The effect of this SNP has been investigated in many previous studies with inconsistent results. The presence of the Ala allele was shown to decrease receptor-mediated transcription activity and to be associated with a lower BMI and increased insulin sensitivity [89]. However, in a recent meta-analysis that included almost 50,000 subjects, the authors showed that individuals carrying the Ala allele have an increased BMI (+0.065 kg/m2), with a stronger effect in Caucasians [90].
Although it seems that PPARγ has a role in ROS clearance from adipose tissue, its paradoxical function has yet to be investigated, to clarify whether the activation of PPARγ decreases ROS production or increases ROS scavenging. Moreover, the association of the Ala allele with obesity needs to be clarified in a controlled experimental setting while carefully studying ROS production.
6.2. PGC1α
PGC1α is a transcriptional co-activator of PPAR α and γ and controls mitochondrial biogenesis, adaptive thermogenesis, oxidative metabolism and glucose homeostasis. In these ways, it increases the oxidative metabolism that will lead to oxidative stress. However, PGC1α also induces the expression of ROS detoxifying enzymes, thus allowing for enhanced oxidative metabolism while controlling the associated ROS production [47,48].
The PGC1α locus harbors the SNP, rs8192678, which results in an amino acid substitution of glycine to serine at position 482 (Gly482Ser) (Figure 2, Table 2). Fanelli et al. found that the Gly482Ser variant was associated with HOMA-IR in obese non-diabetic subjects [91]. In another study, the Gly482Ser variant was significantly associated with a lower BMI, waist and hip circumference and total body fat, but only in women [92]. This SNP was not found to be associated with obesity or type 2 diabetes in overweight non-diabetic Chinese individuals, but it was associated with high insulin, HOMA-IR and waist-hip ratios, as well as with TBARS in hyperglycemia [93]. Because adiponectin is under the transcriptional control of PPARγ, a target of PGC1α, Okauchi et al. studied the effects of the Gly482Ser variant on adiponectin plasma levels and found lower adiponectin concentrations in type 2 diabetic men, but not in women, carrying the polymorphism [94]. In this study, they ruled out the possibility that this variant is a functional polymorphism, and they suggested that the causative SNP could be in linkage disequilibrium (LD) with the common Gly482Ser variant. Along these lines, a previous functional study had already determined that neither the Gly482Ser nor Trp612Met variants of PGC1α affected the functionality of the protein regarding its co-activator activity on PPARγ2 [121]. A study conducted on the Gly482Ser polymorphism showed a basal association for the Ser-Ser genotype with higher HOMA-IR and insulin concentrations, but an intervention with an eight-week low-calorie diet reduced the risk level to that of non-carriers [95].
These results indicate that the Gly482Ser variant has an effect on obesity-associated comorbidities, such as insulin resistance, although it is most likely another SNP that is responsible for these effects.
6.3. NRF2
The transcription factor NRF2 regulates cellular responses to oxidative stress and other endogenous and exogenous stresses. Its role in obesity, type 2 diabetes and metabolic syndrome has already been investigated in many studies using animal models, as reviewed in [122]. NRF2 is regulated mainly through its binding to Kelch-like ECH-associated protein 1 (KEAP1) in the cytoplasm, which leads to its proteasomal degradation. The NRF2/KEAP1 pathway responds to oxidative stress via the control of several antioxidant defense gene expressions harboring the antioxidant response element (ARE) sequence in their promoter (Figure 2).
Along with its role in the response against oxidative stress, NRF2 also modulates adiposity and adipogenesis. In fact, protein levels of NADPH quinone oxidoreductase (NQO1), which is under the transcriptional control of NRF2, increase during the initial stages of the adipogenic differentiation process (days 1–3). Moreover, in addition to NQO1, NRF2 and KEAP1 mRNA levels are also increased in differentiated adipocytes (days 11–14) [123]. This finding has been further confirmed by Hou et al., who found that the lack of NRF2 in 3T3-L1 cells blocked adipogenic differentiation by suppressing CCAAT/enhancer-binding protein beta (CEBPβ) expression, which is needed to trigger the differentiation process [124]. In contrast, Chartoumpekis et al. observed lower NRF2 abundance in the nucleus during adipogenesis, which they hypothesized could lead to the higher ROS levels needed for the differentiation process [125].
Among the in vivo studies on the role of NRF2 in obesity, the main conclusion from knock-out studies in mice is that the targeted disruption of NRF2 decreases adipose tissue mass and protects mice from long-term HFD-induced obesity [126,127]. NRF2 knock-out mice are partially protected from HFD-induced obesity and insulin resistance. This effect may be due to the effect of fibroblast growth factor 21 (FGF21); the mRNA levels of FGF21 in the liver and white adipose tissue were elevated in NRF2 knock-out mice. The opposite effect, i.e., lower FGF21 mRNA levels, was observed when NRF2 was overexpressed [126]. In another study, Shin et al. observed the same effects of the NRF2 knock-out. However, they also tested the pharmacological activation of NRF2 by 2-cyano-3,12-dioxooleana-1,9-dien-28-imidazolide (CDDO-Im) and observed that it also protected from obesity by facilitating higher energy expenditure [128]. One of the main questions arising from these findings concerns which tissue is responsible for the NRF2 effect. One experiment with myeloid cells showed that deficiency in this tissue did not protect mice from HFD-induced adipose tissue inflammation and insulin resistance [129]. As Chartoumpekis et al. state in their review, Cre-loxP system experiments with tissue-specific knock-out models would help further clarify this issue. To our knowledge, only one study of NRF2 in humans has been published, demonstrating how the NRF2 pathway was enriched in individuals with high-fat percentages [130].
Many SNPs have been described for the NRF2 gene in both mice and humans, although the association of NRF2 SNPs and obesity has not been studied. However, other diseases, such as pulmonary disease, breast cancer and gastrointestinal and autoimmune disorders, have shown different associations with NRF2 SNPs [131]. The SNP at position −178C/A conferred lower promoter activity in carriers of the A allele [132]. Interestingly, all evidence indicates that promoter and intronic SNPs are the ones that most show associations with the conditions studied, with no exonic SNP described so far.
Go to:
7. Conclusions
The study of genetic variants in antioxidant defense genes, as well as in the genes of enzymes involved in the generation of ROS, could assist in better understanding the role of antioxidant defenses in protecting against obesity and its derived metabolic complications. There are data supporting the fact that obesity occurs along with enhanced ROS production, either due to misbalanced ROS scavenging systems or to enhanced oxidative stress production in cells. These effects can be due to excessive caloric intake and the saturation of the electron transport chain, as well as to free radical generation from cellular systems, such as the NADPH oxidase complex, in response to the altered insulin or cytokine production that is characteristic of obesity. However, it is becoming clear that ROS themselves can act by increasing adipogenesis and, thus, helping in the development of obesity. Although the reality is most probably a mixture of these phenomena, further studies should be carried out to define the role of ROS in the early stages of the development of obesity and its metabolic alterations, such as insulin resistance.
This review summarizes the studies that have been carried out to unravel the role of genetic variants in antioxidant defense enzymes and other important oxidative metabolism mediators in increasing the risk of obesity or its close comorbidities, such as insulin resistance. It must be stated that many of the association studies reviewed here need validation in independent candidate gene studies or GWAS.
In addition, more studies investigating genetic variations in antioxidant defense system genes are needed to clarify the associations reviewed here, as well as more functional studies concerning these SNPs and their possible impact on enzyme expression levels and activities.
Go to:
Acknowledgments
The present study was funded by the Instituto de Salud Carlos III-Fondo de Investigación Sanitaria (FIS) (project number: PI11/02042 and PI05/1968); Redes temáticas de investigación cooperativa (RETIC), Research Network on Maternal and Child Health and Development (SAMID) RD08/0072/0028 and SAMID RD12/0026/0015; Junta de Andalucía, Consejería de Innovación y Ciencia (project number: P06-CTS 2203 and PI-0296/2007). Rupérez A.I was funded by a Formación de Profesorado Universitario (FPU) stipend from the Ministry of Education and Science of the Spanish Government (AP2009-0547).
Go to:
Conflicts of Interest
The authors declare no conflict of interest.
Go to:
References
1. Shamseddeen H., Getty J.Z., Hamdallah I.N., Ali M.R. Epidemiology and economic impact of obesity and type 2 diabetes. Surg. Clin. N. Am. 2011;91:1163–1172. [PubMed]
2. Gough D.R., Cotter T.G. Hydrogen peroxide: a Jekyll and Hyde signalling molecule. Cell Death Dis. 2011 doi: 10.1038/cddis.2011.96. [PMC free article] [PubMed] [Cross Ref]
3. Da Costa L.A., Badawi A., El–Sohemy A. Nutrigenetics and modulation of oxidative stress. Ann. Nutr. Metab. 2012;60:27–36. [PubMed]
4. Chang Y.C., Yu Y.H., Shew J.Y., Lee W.J., Hwang J.J., Chen Y.H., Chen Y.R., Wei P.C., Chuang L.M., Lee W.H. Deficiency of NPGPx, an oxidative stress sensor, leads to obesity in mice and human. EMBO Mol. Med. 2013;5:1165–1179. [PMC free article] [PubMed]
5. Balistreri C.R., Caruso C., Candore G. The role of adipose tissue and adipokines in obesity-related inflammatory diseases. Mediators Inflamm. 2010 doi: 10.1155/2010/802078. [PMC free article] [PubMed] [Cross Ref]
6. Trayhurn P., Wood I.S. Adipokines: Inflammation and the pleiotropic role of white adipose tissue. Br. J. Nutr. 2004;92:347–355. [PubMed]
7. Bondia-Pons I., Ryan L., Martinez J.A. Oxidative stress and inflammation interactions in human obesity. J. Physiol. Biochem. 2012;68:701–711. [PubMed]
8. Trayhurn P. Hypoxia and adipose tissue function and dysfunction in obesity. Physiol. Rev. 2013;93:1–21. [PubMed]
9. Furukawa S., Fujita T., Shimabukuro M., Iwaki M., Yamada Y., Nakajima Y., Nakayama O., Makishima M., Matsuda M., Shimomura I. Increased oxidative stress in obesity and its impact on metabolic syndrome. J. Clin. Invest. 2004;114:1752–1761. [PMC free article] [PubMed]
10. Anderson E.J., Lustig M.E., Boyle K.E., Woodlief T.L., Kane D.A., Lin C.T., Price J.W., 3rd, Kang L., Rabinovitch P.S., Szeto H.H., et al. Mitochondrial H2O2 emission and cellular redox state link excess fat intake to insulin resistance in both rodents and humans. J. Clin. Invest. 2009;119:573–581. [PMC free article] [PubMed]
11. Bajnok L., Seres I., Varga Z., Jeges S., Peti A., Karanyi Z., Juhasz A., Csongradi E., Mezosi E., Nagy E.V., et al. Relationship of endogenous hyperleptinemia to serum paraoxonase 1, cholesteryl ester transfer protein, and lecithin cholesterol acyltransferase in obese individuals. Metabolism. 2007;56:1542–1549. [PubMed]
12. Keaney J.F., Jr., Larson M.G., Vasan R.S., Wilson P.W., Lipinska I., Corey D., Massaro J.M., Sutherland P., Vita J.A., Benjamin E.J. Framingham study: Obesity and systemic oxidative stress: Clinical correlates of oxidative stress in the Framingham Study. Arterioscler. Thromb. Vasc. Biol. 2003;23:434–439. [PubMed]
13. Codoñer-Franch P., Tavárez-Alonso S., Murria-Estal R., Megías-Vericat J., Tortajada-Girbés M., Alonso-Iglesias E. Nitric oxide production is increased in severely obese children and related to markers of oxidative stress and inflammation. Atherosclerosis. 2011;2215:475–480. [PubMed]
14. Ozgen I.T., Tascilar M.E., Bilir P., Boyraz M., Guncikan M.N., Akay C., Dundaroz R. Oxidative stress in obese children and its relation with insulin resistance. J. Pediatr. Endocrinol. Metab. 2012;25:261–266. [PubMed]
15. Rupérez A.I., Olza J., Gil-Campos M., Leis R., Mesa M.D., Tojo R., Cañete R., Gil A., Aguilera C.M. Are Catalase −844A/G Polymorphism and Activity Associated with Childhood Obesity? Antioxid. Redox Signal. 2013;19:1970–1975. [PMC free article] [PubMed]
16. D’Archivio M., Annuzzi G., Varì R., Filesi C., Giacco R., Scazzocchio B., Santangelo C., Giovannini C., Rivellese A.A., Masella R. Predominant role of obesity/insulin resistance in oxidative stress development. Eur. J. Clin. Invest. 2012;42:70–78. [PubMed]
17. Block G., Dietrich M., Norkus E.P., Morrow J.D., Hudes M., Caan B., Packer L. Factors associated with oxidative stress in human populations. Am. J. Epidemiol. 2002;156:274–285. [PubMed]
18. Meigs J.B., Larson M.G., Fox C.S., Keaney J.F., Jr., Vasan R.S., Benjamin E.J. Association of oxidative stress, insulin resistance, and diabetes risk phenotypes: The Framingham Offspring Study. Diabetes Care. 2007;30:2529–2535. [PubMed]
19. Karbownik-Lewinska M., Szosland J., Kokoszko-Bilska A., Stępniak J., Zasada K., Gesing A., Lewinski A. Direct contribution of obesity to oxidative damage to macromolecules. Neuro Endocrinol. Lett. 2012;33:453–461. [PubMed]
20. Sankhla M., Sharma T.K., Mathur K., Rathor J.S., Butolia V., Gadhok A.K., Vardey S.K., Sinha M., Kaushik G.G. Relationship of oxidative stress with obesity and its role in obesity induced metabolic syndrome. Clin. Lab. 2012;58:385–392. [PubMed]
21. Holvoet P., Lee D.H., Steffes M., Gross M., Jacobs D.R., Jr. Association between circulating oxidized low-density lipoprotein and incidence of the metabolic syndrome. JAMA. 2008;299:2287–2293. [PMC free article] [PubMed]
22. Van Guilder G.P., Hoetzer G.L., Greiner J.J., Stauffer B.L., Desouza C.A. Influence of metabolic syndrome on biomarkers of oxidative stress and inflammation in obese adults. Obesity (Silver Spring) 2006;14:2127–2231. [PubMed]
23. Aslan M., Horoz M., Sabuncu T., Celik H., Selek S. Serum paraoxonase enzyme activity and oxidative stress in obese subjects. Pol. Arch. Med. Wewn. 2011;121:181–185. [PubMed]
24. Ferretti G., Bacchetti T., Moroni C., Savino S., Liuzzi A., Balzola F., Bicchiega V. Paraoxonase activity in high-density lipoproteins: A comparison between healthy and obese females. J. Clin. Endocrinol. Metab. 2005;90:1728–1733. [PubMed]
25. Martínez-Salazar M.F., Almenares-López D., García-Jiménez S., Sánchez-Alemán M.A., Juantorena-Ugás A., Ríos C., Monroy-Noyola A. Relationship between the paraoxonase (PON1) L55M and Q192R polymorphisms and obesity in a Mexican population: A pilot study. Genes Nutr. 2011;6:361–368. [PMC free article] [PubMed]
26. Veiga L., Silva-Nunes J., Melão A., Oliveira A., Duarte L., Brito M. Q192R polymorphism of the paraoxonase-1 gene as a risk factor for obesity in Portuguese women. Eur. J. Endocrinol. 2011;164:213–218. [PubMed]
27. Tabur S., Torun A.N., Sabuncu T., Turan M.N., Celik H., Ocak A.R., Aksoy N. Non-diabetic metabolic syndrome and obesity do not affect serum paraoxonase and arylesterase activities but do affect oxidative stress and inflammation. Eur. J. Endocrinol. 2010;162:535–541. [PubMed]
28. Krzystek-Korpacka M., Patryn E., Boehm D., Berdowska I., Zielinski B., Noczynska A. Advanced oxidation protein products (AOPPs) in juvenile overweight and obesity prior to and following weight reduction. Clin. Biochem. 2008;41:943–949. [PubMed]
29. Warolin J., Coenen K.R., Kantor J.L., Whitaker L.E., Wang L., Acra S.A., Roberts L.J., 2nd, Buchowski M.S. The relationship of oxidative stress, adiposity and metabolic risk factors in healthy Black and White American youth. Pediatr. Obes. 2014;9:43–52. [PMC free article] [PubMed]
30. Oliver S.R., Rosa J.S., Milne G.L., Pontello A.M., Borntrager H.L., Heydari S., Galassetti P.R. Increased oxidative stress and altered substrate metabolism in obese children. Int. J. Pediatr. Obes. 2010;5:436–444. [PMC free article] [PubMed]
31. Araki S., Dobashi K., Yamamoto Y., Asayama K., Kusuhara K. Increased plasma isoprostane is associated with visceral fat, high molecular weight adiponectin, and metabolic complications in obese children. Eur. J. Pediatr. 2010;169:965–970. [PubMed]
32. Codoñer-Franch P., Tavárez-Alonso S., Porcar-Almela M., Navarro-Solera M., Arilla-Codoñer A., Alonso-Iglesias E. Plasma resistin levels are associated with homocysteine, endothelial activation, and nitrosative stress in obese youths. Clin. Biochem. 2014;47:44–48. [PubMed]
33. Kelly A.S., Jacobs D.R., Jr., Sinaiko A.R., Moran A., Steffen L.M., Steinberger J. Relation of circulating oxidized LDL to obesity and insulin resistance in children. Pediatr. Diabetes. 2010;11:552–555. [PMC free article] [PubMed]
34. Koncsos P., Seres I., Harangi M., Illyés I., Józsa L., Gönczi F., Bajnok L., Paragh G. Human paraoxonase-1 activity in childhood obesity and its relation to leptin and adiponectin levels. Pediatr. Res. 2010;67:309–313. [PubMed]
35. Krzystek-Korpacka M., Patryn E., Hotowy K., Czapińska E., Majda J., Kustrzeba-Wójcicka I., Noczyńska A., Gamian A. Paraoxonase (PON)-1 activity in overweight and obese children and adolescents: Association with obesity-related inflammation and oxidative stress. Adv. Clin. Exp. Med. 2013;22:229–236. [PubMed]
36. Ferré N., Feliu A., García-Heredia A., Marsillach J., París N., Zaragoza-Jordana M., Mackness B., Mackness M., Escribano J., Closa-Monasterolo R., et al. Impaired paraoxonase-1 status in obese children. Relationships with insulin resistance and metabolic syndrome. Clin. Biochem. 2013;46:1830–1836. [PubMed]
37. Rupérez A.I., López-Guarnido O., Gil F., Olza J., Gil-Campos M., Leis R., Tojo R., Cañete R., Gil A., Aguilera C.M. Paraoxonase 1 activities and genetic variation in childhood obesity. Br. J. Nutr. 2013;110:1639–1647. [PubMed]
38. Pirgon Ö., Bilgin H., Çekmez F., Kurku H., Dündar B.N. Association between insulin resistance and oxidative stress parameters in obese adolescents with non-alcoholic fatty liver disease. J. Clin. Res. Pediatr. Endocrinol. 2013;5:33–39. [PMC free article] [PubMed]
39. Krieger-Brauer H.I., Medda P.K., Kather H. The stimulus-sensitive H2O2-generating system present in human fat-cell plasma membranes is multireceptor-linked and under antagonistic control by hormones and cytokines. Biochem. J. 1995;307:543–548. [PMC free article] [PubMed]
40. Finkel T. Signal transduction by reactive oxygen species. J. Cell Biol. 2006;194:7–15. [PMC free article] [PubMed]
41. Houstis N., Rosen E.D., Lander E.S. Reactive oxygen species have a causal role in multiple forms of insulin resistance. Nature. 2006;440:944–948. [PubMed]
42. Styskal J., Nwagwu F.A., Watkins Y.N., Liang H., Richardson A., Musi N., Salmon A.B. Methionine sulfoxide reductase A affects insulin resistance by protecting insulin receptor function. Free Radic. Biol. Med. 2013;56:123–132. [PMC free article] [PubMed]
43. Gregor M.F., Hotamisligil G.S. Inflammatory mechanisms in obesity. Annu. Rev. Immunol. 2011;29:415–545. [PubMed]
44. Huh J.Y., Kim Y., Jeong J., Park J., Kim I., Huh K.H., Kim Y.S., Woo H.A., Rhee S.G., Lee K.J., et al. Peroxiredoxin 3 is a key molecule regulating adipocyte oxidative stress, mitochondrial biogenesis, and adipokine expression. Antioxid. Redox Signal. 2012;16:229–243. [PMC free article] [PubMed]
45. Luo W., Cao J., Li J., He W. Adipose tissue-specific PPARγ deficiency increases resistance to oxidative stress. Exp. Gerontol. 2008;43:154–163. [PubMed]
46. Itoh H., Doi K., Tanaka T., Fukunaga Y., Hosoda K., Inoue G., Nishimura H., Yoshimasa Y., Yamori Y., Nakao K. Hypertension and insulin resistance: Role of peroxisome proliferator-activated receptor gamma. Clin. Exp. Pharmacol. Physiol. 1999;26:558–560. [PubMed]
47. Austin S., St-Pierre J. PGC1a and mitochondrial metabolism - emerging concepts and relevance in ageing and neurodegenerative disorders. J. Cell Sci. 2012;125:4963–4971. [PubMed]
48. Valle I., Alvarez-Barrientos A., Arza E., Lamas S., Monsalve M. PGC-1a regulates the mitochondrial antioxidant defense system in vascular endothelial cells. Cardiovasc. Res. 2005;66:562–573. [PubMed]
49. Margis R., Dunand C., Teixeira F.K., Margis-Pinheiro M. Glutathione peroxidase family - an evolutionary overview. FEBS J. 2008;275:3959–3970. [PubMed]
50. Yoshimura S., Watanabe K., Suemizu H., Onozawa T., Mizoguchi J., Tsuda K., Hatta H., Moriuchi T. Tissue specific expression of the plasma glutathione peroxidase gene in rat kidney. J. Biochem. 1991;109:918–923. [PubMed]
51. Toppo S., Vanin S., Bosello V., Tosatto S.C. Evolutionary and structural insights into the multifaceted glutathione peroxidase (Gpx) superfamily. Antioxid. Redox. Signal. 2008;10:1501–1514. [PubMed]
52. Wei P.C., Hsieh Y.H., Su M.I., Jiang X., Hsu P.H., Lo W.T., Weng J.Y., Jeng Y.M., Wang J.M., Chen P.L., et al. Loss of the oxidative stress sensor NPGPx compromises GRP78 chaperone activity and induces systemic disease. Mol. Cell. 2012;48:747–759. [PMC free article] [PubMed]
53. Asayama K., Nakane T., Dobashi K., Kodera K., Hayashibe H., Uchida N., Nakazawa S. Effect of obesity and troglitazone on expression of two glutathione peroxidases: Cellular and extracellular types in serum, kidney and adipose tissue. Free Radic. Res. 2001;34:337–347. [PubMed]
54. Lijnen H.R., Van Hul M., Hemmeryckx B. Caloric restriction improves coagulation and inflammation profile in obese mice. Thromb. Res. 2012;129:74–79. [PubMed]
55. Loh K., Deng H., Fukushima A., Cai X., Boivin B., Galic S., Bruce C., Shields B.J., Skiba B., Ooms L.M., et al. Reactive oxygen species enhance insulin sensitivity. Cell Metab. 2009;10:260–272. [PMC free article] [PubMed]
56. Merry T.L., Tran M., Stathopoulos M., Wiede F., Fam B.C., Dood G.T., Clarke I., Watt M.J., Andrikopoulos S., Tiganis T. High fat fed obese Gpx1-deficient mice exhibit defective insulin secretion but protection from hepatic steatosis and liver damage. Antioxid. Redox Signal. 2013 doi: 10.1089/ars.2013.5428. [PubMed] [Cross Ref]
57. De Haan J.B., Witting P.K., Stefanovic N., Pete J., Daskalakis M., Kola I., Stocker R., Smolich J.J. Lack of the antioxidant glutathione peroxidase-1 does not increase atherosclerosis in C57BL/J6 mice fed a high-fat diet. J. Lipid Res. 2006;47:1157–1167. [PubMed]
58. Lee Y.S., Kim A.Y., Choi J.W., Kim M., Yasue S., Son H.J., Masuzaki H., Park K.S., Kim J.B. Dysregulation of adipose glutathione peroxidase 3 in obesity contributes to local and systemic oxidative stress. Mol. Endocrinol. 2008;22:2176–2189. [PubMed]
59. Lundholm L., Putnik M., Otsuki M., Andersson S., Ohlsson C., Gustafsson J.A., Dahlman-Wright K. Effects of estrogen on gene expression profiles in mouse hypothalamus and white adipose tissue: target genes include glutathione peroxidase 3 and cell death-inducing DNA fragmentation factor, alpha-subunit-like effector A. J. Endocrinol. 2008;196:547–557. [PubMed]
60. Kuzuya M., Ando F., Iguchi A., Shimokata H. Glutathione peroxidase 1 Pro198Leu variant contributes to the metabolic syndrome in men in a large Japanese cohort. Am. J. Clin. Nutr. 2008;87:1939–1944. [PubMed]
61. Cominetti C., de Bortoli M.C., Purgatto E., Ong T.P., Moreno F.S., Garrido A.B., Jr., Cozzolino S.M. Associations between glutathione peroxidase-1 Pro198Leu polymorphism, selenium status, and DNA damage levels in obese women after consumption of Brazil nuts. Nutrition. 2011;27:891–896. [PubMed]
62. Shuvalova Y.A., Kaminnyi A.I., Meshkov A.N., Kukharchuk V.V. Pro198Leu polymorphism of GPx-1 gene and activity of erythrocytic glutathione peroxidase and lipid peroxidation products. Bull. Exp. Biol. Med. 2010;149:743–745. [PubMed]
63. Hamanishi T., Furuta H., Kato H., Doi A., Tamai M., Shimomura H., Sakagashira S., Nishi M., Sasaki H., Sanke T., et al. Functional variants in the glutathione peroxidase-1 (GPx-1) gene are associated with increased intima-media thickness of carotid arteries and risk of macrovascular diseases in japanese type 2 diabetic patients. Diabetes. 2004;53:2455–2460. [PubMed]
64. Rindler P.M., Plafker S.M., Szweda L.I., Kinter M. High dietary fat selectively increases catalase expression within cardiac mitochondria. J. Biol. Chem. 2013;288:1979–1990. [PMC free article] [PubMed]
65. Shin M.J., Park E. Contribution of insulin resistance to reduced antioxidant enzymes and vitamins in nonobese Korean children. Clin. Chim. Acta. 2006;365:200–205. [PubMed]
66. Wang Z., Li Y., Wang B., He Y., Wang Y., Xi H., Li Y., Wang Y., Zhu D., Jin J., et al. A haplotype of the catalase gene confers an increased risk of essential hypertension in Chinese Han. Hum. Mutat. 2010;31:272–278. [PubMed]
67. Adkins S., Gan K.N., Mody M., La Du B.N. Molecular basis for the polymorphic forms of human serum paraoxonase/arylesterase: Glutamine or arginine at position 191, for the respective A or B allozymes. Am. J. Hum. Genet. 1993;52:598–608. [PMC free article] [PubMed]
68. Baráth A., Németh I., Karg E., Endreffy E., Bereczki C., Gellén B., Haszon I., Túri S. Roles of paraoxonase and oxidative stress in adolescents with uraemic, essential or obesity-induced hypertension. Kidney Blood Press. Res. 2006;29:144–151. [PubMed]
69. Hiroi M., Nagahara Y., Miyauchi R., Misaki Y., Goda T., Kasezawa N., Sasaki S., Yamakawa-Kobayashi K. The combination of genetic variations in the PRDX3 gene and dietary fat intake contribute to obesity risk. Obesity (Silver Spring) 2011;19:882–887. [PubMed]
70. Lindgren C.M., Heid I.M., Randall J.C., Lamina C., Steinthorsdottir V., Qi L., Speliotes E.K., Thorleifsson G., Willer C.J., Herrera B.M., et al. Genome-wide association scan meta-analysis identifies three Loci influencing adiposity and fat distribution. PLoS Genet. 2009;5:e1000508. [PMC free article] [PubMed]
71. Bille D.S., Banasik K., Justesen J.M., Sandholt C.H., Sandbæk A., Lauritzen T., Jørgensen T., Witte D.R., Holm J.C., Hansen T., et al. Implications of central obesity-related variants in LYPLAL1, NRXN3, MSRA, and TFAP2B on quantitative metabolic traits in adult Danes. PLoS One. 2011;6:e20640. [PMC free article] [PubMed]
72. Graff M., Fernández-Rhodes L., Liu S., Carlson C., Wassertheil-Smoller S., Neuhouser M., Reiner A., Kooperberg C., Rampersaud E., Manson J.E., et al. Generalization of adiposity genetic loci to US Hispanic women. Nutr. Diabetes. 2013;3:e85. [PMC free article] [PubMed]
73. Yeung E., Qi L., Hu F.B., Zhang C. Novel abdominal adiposity genes and the risk of type 2 diabetes: findings from two prospective cohorts. Int. J. Mol. Epidemiol. Genet. 2011;2:138–144. [PMC free article] [PubMed]
74. Delahanty R.J., Beeghly-Fadiel A., Xiang Y.B., Long J., Cai Q., Wen W., Xu W.H., Cai H., He J., Gao Y.T., Zheng W., Shu X.O. Association of obesity-related genetic variants with endometrial cancer risk: A report from the Shanghai Endometrial Cancer Genetics Study. Am. J. Epidemiol. 2011;174:1115–1126. [PMC free article] [PubMed]
75. Hotta K., Kitamoto T., Kitamoto A., Mizusawa S., Matsuo T., Nakata Y., Kamohara S., Miyatake N., Kotani K., Komatsu R., et al. Association of variations in the FTO, SCG3 and MTMR9 genes with metabolic syndrome in a Japanese population. J. Hum. Genet. 2011;56:647–651. [PubMed]
76. Hotta K., Nakamura M., Nakamura T., Matsuo T., Nakata Y., Kamohara S., Miyatake N., Kotani K., Komatsu R., Itoh N., et al. Polymorphisms in NRXN3, TFAP2B, MSRA, LYPLAL1, FTO and MC4R and their effect on visceral fat area in the Japanese population. J. Hum. Genet. 2010;55:738–742. [PubMed]
77. Scherag A., Dina C., Hinney A., Vatin V., Scherag S., Vogel C.I., Müller T.D., Grallert H., Wichmann H.E., Balkau B., et al. Two new Loci for body-weight regulation identified in a joint analysis of genome-wide association studies for early-onset extreme obesity in French and german study groups. PLoS Genet. 2010;6:e1000916. [PMC free article] [PubMed]
78. Dorajoo R., Blakemore A.I., Sim X., Ong R.T., Ng D.P., Seielstad M., Wong T.Y., Saw S.M., Froguel P., Liu J., et al. Replication of 13 obesity loci among Singaporean Chinese, Malay and Asian-Indian populations. Int. J. Obes. (Lond) 2012;36:159–163. [PubMed]
79. Scherag A., Kleber M., Boes T., Kolbe A.L., Ruth A., Grallert H., Illig T., Heid I.M., Toschke A.M., Grau K., et al. SDCCAG8 obesity alleles and reduced weight loss after a lifestyle intervention in overweight children and adolescents. Obesity (Silver Spring) 2012;20:466–470. [PubMed]
80. Sutton A., Khoury H., Prip-Buus C., Cepanec C., Pessayre D., Degoul F. The Ala16Val genetic dimorphism modulates the import of human manganese superoxide dismutase into rat liver mitochondria. Pharmacogenetics. 2003;13:145–157. [PubMed]
81. Montano M.A., Barrio Lera J.P., Gottlieb M.G., Schwanke C.H., da Rocha M.I., Manica-Cattani M.F., dos Santos G.F., da Cruz I.B. Association between manganese superoxide dismutase (MnSOD) gene polymorphism and elderly obesity. Mol. Cell. Biochem. 2009;328:33–40. [PubMed]
82. Montano M.A., da Cruz I.B., Duarte M.M., da Krewer C.C., da Rocha M.I., Mânica-Cattani M.F., Soares F.A., Rosa G., Maris A.F., Battiston F.G., et al. Inflammatory cytokines in vitro production are associated with Ala16Val superoxide dismutase gene polymorphism of peripheral blood mononuclear cells. Cytokine. 2012;60:30–33. [PubMed]
83. Caple F., Williams E.A., Spiers A., Tyson J., Burtle B., Daly A.K., Mathers J.C., Hesketh J.E. Inter- individual variation in DNA damage and base excision repair in young, healthy nonsmokers: Effects of dietary supplementation and genotype. Br. J. Nutr. 2010;103:1585–1593. [PubMed]
84. San José G., Moreno M.U., Olivan S., Beloqui O., Fortuno A., Díez J., Zalba G. Functional effect of the p22phox −930A/G polymorphism on p22phox expression and NADPH oxidase activity in hypertension. Hypertension. 2004;44:163–169. [PubMed]
85. Ochoa M.C., Razquin C., Zalba G., Martínez-González M.A., Martínez J.A., Marti A. G allele of the -930A>G polymorphism of the CYBA gene is associated with insulin resistance in obese subjects. J. Physiol. Biochem. 2008;64:127–133. [PubMed]
86. Hayaishi-Okano R., Yamasaki Y., Kajimoto Y., Sakamoto K., Ohtoshi K., Katakami N., Kawamori D., Miyatsuka T., Hatazaki M., Hazama Y., et al. Association of NAD(P)H oxidase p22 phox gene variation with advanced carotid atherosclerosis in Japanese type 2 diabetes. Diabetes Care. 2003;26:458–463. [PubMed]
87. Schreiber R., Ferreira-Sae M.C., Tucunduva A.C., Mill J.G., Costa F.O., Krieger J.E., Franchini K.G., Pereira A.C., Nadruz W., Jr. CYBA C242T polymorphism is associated with obesity and diabetes mellitus in Brazilian hypertensive patients. Diabet. Med. 2012;29:e55–e61. [PubMed]
88. Kim C., Zheng T., Lan Q., Chen Y., Foss F., Chen X., Holford T., Leaderer B., Boyle P., Chanock S.J., et al. Genetic polymorphisms in oxidative stress pathway genes and modification of BMI and risk of non-Hodgkin lymphoma. Cancer Epidemiol. Biomark. Prev. 2012;21:866–868. [PMC free article] [PubMed]
89. Deeb S.S., Fajas L., Nemoto M., Pihlajamäki J., Mykkänen L., Kuusisto J., Laakso M., Fujimoto W., Auwerx J. A Pro12Ala substitution in PPARgamma2 associated with decreased receptor activity, lower body mass index and improved insulin sensitivity. Nat. Genet. 1998;20:284–287. [PubMed]
90. Galbete C., Toledo E., Martínez-González M.A., Martínez J.A., Guillén-Grima F., Marti A. Pro12Ala variant of the PPARG2 gene increases body mass index: An updated meta-analysis encompassing 49,092 subjects. Obesity (Silver Spring) 2013 doi: 10.1002/oby.20150. [PubMed] [Cross Ref]
91. Fanelli M., Filippi E., Sentinelli F., Romeo S., Fallarino M., Buzzetti R., Leonetti F., Baroni M.G. The Gly482Ser missense mutation of the peroxisome proliferator-activated receptor gamma coactivator-1 alpha (PGC-1 alpha) gene associates with reduced insulin sensitivity in normal and glucose-intolerant obese subjects. Dis. Markers. 2005;21:175–180. [PMC free article] [PubMed]
92. Esterbauer H., Oberkofler H., Linnemayr V., Iglseder B., Hedegger M., Wolfsgruber P., Paulweber B., Fastner G., Krempler F., Patsch W. Peroxisome proliferator-activated receptor-gamma coactivator-1 gene locus: associations with obesity indices in middle-aged women. Diabetes. 2002;51:1281–1286. [PubMed]
93. Weng S.W., Lin T.K., Wang P.W., Chen I.Y., Lee H.C., Chen S.D., Chuang Y.C., Liou C.W. Gly482Ser polymorphism in the peroxisome proliferator-activated receptor gamma coactivator-1alpha gene is associated with oxidative stress and abdominal obesity. Metabolism. 2010;59:581–586. [PubMed]
94. Okauchi Y., Iwahashi H., Okita K., Yuan M., Matsuda M., Tanaka T., Miyagawa J., Funahashi T., Horikawa Y., Shimomura I., et al. PGC-1alpha Gly482Ser polymorphism is associated with the plasma adiponectin level in type 2 diabetic men. Endocr. J. 2008;55:991–997. [PubMed]
95. Goyenechea E., Crujeiras A.B., Abete I., Parra D., Martínez J.A. Enhanced short-term improvement of insulin response to a low-caloric diet in obese carriers the Gly482Ser variant of the PGC-1alpha gene. Diabetes Res. Clin. Pract. 2008;82:190–196. [PubMed]
96. Ahn J., Gammon M.D., Santella R.M., Gaudet M.M., Britton J.A., Teitelbaum S.L., Terry M.B., Nowell S., Davis W., Garza C., et al. Associations between breast cancer risk and the catalase genotype, fruit and vegetable consumption, and supplement use. Am. J. Epidemiol. 2005;162:943–952. [PubMed]
97. Nadif R., Mintz M., Jedlicka A., Bertrand J.P., Kleeberger S.R., Kauffmann F. Association of CAT polymorphisms with catalase activity and exposure to environmental oxidative stimuli. Free Radic. Res. 2005;39:1345–1350. [PMC free article] [PubMed]
98. Bastaki M., Huen K., Manzanillo P., Chande N., Chen C., Balmes J.R., Tager I.B., Holland N. Genotype-activity relationship for Mn-superoxide dismutase, glutathione peroxidases 1 and catalase in humans. Pharmacogenet. Genom. 2006;16:279–286. [PubMed]
99. Forsberg L., Lyrenás L., De Faire U., Morgenstern R. A common functional C-T substitution polymorphism in the promoter region of the human catalase gene influences transcription factor binding, reporter gene transcription and is correlated to blood catalase levels. Free Radic. Biol. Med. 2001;30:500–505. [PubMed]
100. Labrecque B., Beaudry D., Mayhue M., Hallé C., Bordignon V., Murphy B.D., Palin M.F. Molecular characterization and expression analysis of the porcine paraoxonase 3 (PON3) gene. Gene. 2009;443:110–120. [PubMed]
101. Costa L.G., Giordano G., Furlong C.E. Pharmacological and dietary modulators of paraoxonase 1 (PON1) activity and expression: the hunt goes on. Biochem. Pharmacol. 2011;81:337–344. [PMC free article] [PubMed]
102. Garin M.C., James R.W., Dussoix P., Blanché H., Passá P., Froguel P., Ruiz J. Paraoxonase Polymorphism Met-Leu54 is associated with modified serum concentrations of the enzyme. A possible link between the paraoxonase gene and increased risk of cardiovascular disease in diabetes. J. Clin. Invest. 1997;99:62–66. [PMC free article] [PubMed]
103. Cox A.G., Winterbourn C.C., Hampton M.B. Mitochondrial peroxiredoxin involvement in antioxidant defense and redox signaling. Biochem. J. 2010;425:313–325. [PubMed]
104. Zelko I.N., Mariani T.J., Folz R.J. Superoxide dismutase multigene family: A comparison of the CuZn-SOD (SOD1), MN-SOD (SOD2), and EC-SOD (SOD3) gene structures, evolution, and expression. Free Radic. Biol. Med. 2002;33:337–349. [PubMed]
105. Nakao C., Ookawara T., Sato Y., Kizaki T., Imazeki N., Matsubara O., Haga S., Suzuki K., Taniguchi N., Ohno H. Extracellular superoxide dismutase in tissues from obese (ob/ob) mice. Free Radic. Res. 2000;33:229–241. [PubMed]
106. Adachi T., Inoue M., Hara H., Maehata E., Suzuki S. Relationship of plasma extracellular-superoxide dismutase level with insulin resistance in type 2 diabetic patients. J. Endocrinol. 2004;181:413–417. [PubMed]
107. Jang Y.C., Pérez V.I., Song W., Lustgarten M.S., Salmon A.B., Mele J., Qi W., Liu Y., Liang H., Chaudhuri A., et al. Overexpression of Mn superoxide dismutase does not increase life span in mice. J. Gerontol. A Biol. Sci. Med. Sci. 2009;64:1114–1125. [PMC free article] [PubMed]
108. Hoehn K.L., Salmon A.B., Hohnen-Behrens C., Turner N., Hoy A.J., Maghzal G.J., Stocker R., Van Remmen H., Kraegen E.W., Cooney G.J., et al. Insulin resistance is a cellular antioxidant defense mechanism. Proc. Natl. Acad. Sci. USA. 2009;106:17787–17792. [PMC free article] [PubMed]
109. Liu Y., Qi W., Richardson A., van Remmen H., Ikeno Y., Salmon A.B. Oxidative damage associated with obesity is prevented by overexpression of CuZn- or Mn-superoxide dismutase. Biochem. Biophys. Res. Commun. 2013;16:78–83. [PMC free article] [PubMed]
110. Kurahashi T., Konno T., Otsuki N., Kwon M., Tsunoda S., Ito J., Fujii J. A malfunction in triglyceride transfer from the intracellular lipid pool to apoB in enterocytes of SOD1-deficient mice. FEBS Lett. 2012;586:4289–4295. [PubMed]
111. Shimoda-Matsubayashi S., Matsumine H., Kobayashi T., Nakagawa-Hattori Y. Structural dimorphism in the mitochondrial targeting sequence in the human manganese superoxide dismutase gene. Biochem. Biophys. Res. Commun. 1996;226:561–565. [PubMed]
112. El-Koofy N.M., El-Karaksy H.M., Mandour I.M., Anwar G.M., El-Raziky M.S., El-Hennawy A.M. Genetic polymorphisms in non-alcoholic fatty liver disease in obese Egyptian children. Saudi J. Gastroenterol. 2011;17:265–270. [PMC free article] [PubMed]
113. Bertelsen M., Anggard E.E., Carrier M.J. Oxidative stress impairs insulin internalization inendothelial cells in vitro. Diabetologia. 2001;44:605–613. [PubMed]
114. Tirosh A., Potashnik R., Bashan N., Rudich A. Oxidative stress disrupts insulin-induced cellular redistribution of insulin receptor substrate-1 and phosphatidylinositol 3-kinase in 3T3-L1 adipocytes: A putative cellular mechanism for impaired proteinkinase B activation and GLUT4 translocation. J. Biol.Chem. 1999;274:10595–10602. [PubMed]
115. Uthus E.O., Picklo M.J., Sr. Obesity reduces methionine sulphoxide reductase activity in visceral adipose tissue. Free Radic. Res. 2011;45:1052–1060. [PubMed]
116. DeCoursey T.E., Ligeti E. Regulation and termination of NADPH oxidase activity. Cell. Mol. Life Sci. 2005;62:2173–2193. [PubMed]
117. Eriksson J.W. Metabolic stress in insulin’s target cells leads to ROS accumulation—A hypothetical common pathway causing insulin resistance. FEBS Lett. 2007;581:3734–3742. [PubMed]
118. Vidal-Puig A.J., Considine R.V., Jimenez-Liñan M., Werman A., Pories W.J., Caro J.F., Flier J.S. Peroxisome proliferator-activated receptor gene expression in human tissues. Effects of obesity, weight loss, and regulation by insulin and glucocorticoids. J. Clin. Invest. 1997;99:2416–2422. [PMC free article] [PubMed]
119. Berhouma R., Kouidhi S., Ammar M., Abid H., Ennafaa H., Benammar-Elgaaied A. Correlation of peroxisome proliferator-activated receptor (PPAR-γ) mRNA expression with Pro12Ala polymorphism in obesity. Biochem. Genet. 2013;51:256–263. [PubMed]
120. Zielekiak A., Wójcik M., Woźniak L.A. Structure and physiological functions of the human peroxisome proliferator-activated receptor γ Arch. Immunol. Ther. Exp. 2008;56:331–345. [PubMed]
121. Nitz I., Ewert A., Klapper M., Döring F. Analysis of PGC-1alpha variants Gly482Ser and Thr612Met concerning their PPARgamma2-coactivation function. Biochem. Biophys. Res. Commun. 2007;353:481–486. [PubMed]
122. Chartoumpekis D.V., Kensler T.W. New player on an old field; the Keap1/Nrf2 pathway as a target for treatment of type 2 diabetes and metabolic syndrome. Curr. Diabetes Rev. 2013;9:137–145. [PMC free article] [PubMed]
123. Vomhof-DeKrey E.E., Picklo M.J. NAD(P)H:quinone oxidoreductase 1 activity reduces hypertrophy in 3T3-L1 adipocytes. Free Radic. Biol. Med. 2012;53:690–700. [PubMed]
124. Hou Y., Xue P., Bai Y., Liu D., Woods C.G., Yarborough K., Fu J., Zhang Q., Sun G., Collins S., et al. Nuclear factor erythroid-derived factor 2-related factor 2 regulates transcription of CCAAT/enhancer-binding protein β during adipogenesis. Free Radic. Biol. Med. 2012;52:462–472. [PMC free article] [PubMed]
125. Chartoumpekis D.V., Ziros P.G., Sykiotis G.P., Zaravinos A., Psyrogiannis A.I., Kyriazopoulou V.E., Spandidos D.A., Habeos I.G. Nrf2 activation diminishes during adipocyte differentiation of ST2 cells. Int. J. Mol. Med. 2011;28:823–238. [PubMed]
126. Chartoumpekis D.V., Ziros P.G., Psyrogiannis A.I., Papavassiliou A.G., Kyriazopoulou V.E., Sykiotis G.P., Habeos I.G. Nrf2 represses FGF21 during long-term high-fat diet-induced obesity in mice. Diabetes. 2011;60:2465–2473. [PMC free article] [PubMed]
127. Pi J., Leung L., Xue P., Wang W., Hou Y., Liu D., Yehuda-Shnaidman E., Lee C., Lau J., Kurtz T.W., et al. Deficiency in the nuclear factor E2-related factor-2 transcription factor results in impaired adipogenesis and protects against diet-induced obesity. J. Biol. Chem. 2010;285:9292–9300. [PMC free article] [PubMed]
128. Shin S., Wakabayashi J., Yates M.S., Wakabayashi N., Dolan P.M., Aja S., Liby K.T., Sporn M.B., Yamamoto M., Kensler T.W. Role of Nrf2 in prevention of high-fat diet-induced obesity by synthetic triterpenoid CDDO-imidazolide. Eur. J. Pharmacol. 2009;620:138–144. [PMC free article] [PubMed]
129. Meher A.K., Sharma P.R., Lira V.A., Yamamoto M., Kensler T.W., Yan Z., Leitinger N. Nrf2 deficiency in myeloid cells is not sufficient to protect mice from high-fat diet-induced adipose tissue inflammation and insulin resistance. Free Radic. Biol. Med. 2012;52:1708–1715. [PMC free article] [PubMed]
130. Das S.K., Sharma N.K., Hasstedt S.J., Mondal A.K., Ma L., Langberg K.A., Elbein S.C. An integrative genomics approach identifies activation of thioredoxin/thioredoxin reductase-1-mediated oxidative stress defense pathway and inhibition of angiogenesis in obese nondiabetic human subjects. J. Clin. Endocrinol. Metab. 2011;96:E1308–E1313. [PMC free article] [PubMed]
131. Cho H.Y. Genomic Structure Variation of Nuclear Factor (Erythroid-Derived 2)-Like 2. Oxid. Med. Cell. Longev. 2013 doi: 10.1155/2013/286524. [PMC free article] [PubMed] [Cross Ref]
132. Marzec J.M., Christie J.D., Reddy S.P., Jedlicka A.E., Vuong H., Lanken P.N., Aplenc R., Yamamoto T., Yamamoto M., Cho H.Y., Kleeberger S.R. Functional polymorphisms in the transcription factor NRF2 in humans increase the risk of acute lung injury. FASEB J. 2007;21:2237–2246.